Cellular, molecular, genomic changes occurring in the heart under mechanical circulatory support
Introduction
Heart failure (HF) is a clinical syndrome that arises from any functional or structural impairment of one or two ventricles in either filling or ejection of blood. The clinical syndrome of HF results from disorders of the pericardium, myocardium, endocardium, heart valves or metabolic abnormalities, and manifests itself mainly as impaired left ventricular (LV) myocardial function (1).
The clinical syndrome of HF is paralleled by significant molecular, cellular and histologic changes, known as “cardiac remodelling”. The long waiting times for donor organs and the need for treatment of end-stage HF unresponsive to medical therapy has required the development of LV assist devices (LVAD). LVADs provide volume and pressure unloading of the LV and restore function and allow myocardial recovery. Ventricular unloading enables a reversal of stress-related compensatory responses of the overloaded myocardium and normalizes systemic perfusion of organs. Thus, ventricular unloading activates a local and systemic neurohormonal and cytokine network and is associated with morphological and molecular changes in the myocardium, called “reverse remodelling”.
However, molecular reverse remodelling does not always correspond to functional myocardial recovery, and in this case LVAD will act as a bridge to heart transplantation or to destination therapy. It is important to consider the etiology of HF to predict the recovery and reverse remodelling of the myocardium. For example, primary cardiomyopathy, which has a genetic etiology with an altered proteomic pattern, cannot undergo to myocardial recovery. We review the cellular, molecular and genetic changes during LV unloading (Figure 1).
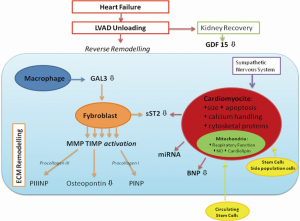
Cardiomyocyte
Morphology and contractility
The unloading achieved with LVADs results in a reduction in the LV dilation seen in end-stage HF and several studies have shown that prolonged mechanical support leads to decreased LV diastolic diameter, LV end-diastolic pressure, pulmonary capillary wedge pressure, and pulmonary vascular resistance, as well as increased ejection fraction (EF), mean aortic pressure, and cardiac index (2,3).
In a study of myocytes obtained from patients with HF [etiology was both ischemic cardiomyopathy (ICD) and dilated cardiomyopathy (DCD)] at the time of transplant, the myocytes from patients supported with an LVAD had a greater degree of shortening, faster time to peak contraction and faster time to shortening (4). Another study obtained in DCM demonstrated a significant improvement in LV and myocyte size during LVAD support, but there was only partial recovery of EF and myocyte contractility (5). These conflicting results may suggest that the myocyte recovery is pathology-related: primary cardiomyopathy with an altered proteomic pattern presents as a congenitally impaired myocardial contraction system.
Mechanical unloading has also been associated with a specific pattern of changes in sarcomeric, non-sarcomeric and membrane-associated proteins. These changes in expression of cytoskeletal proteins require further study to better explain the differences in myocyte contractility seen with different etiologies of cardiomyopathy (6).
Calcium handling
HF is associated with alterations of Ca2+ cycling. Downregulated gene expression of sarcoplasmic endoreticular Ca2+ ATPase subtype 2a (SERCA2a), of the sarcoplasmic reticular (SR) ryanodine-sensitive Ca2+ release channel [ryanodine receptor (RyR)], and altered regulation of the sarcolemmal Na+/Ca2+ exchanger (NCX1) have been reported and appear to correlate with contractile dysfunction (7).
After LVAD support, NCX1 and SERCA2a mRNA was upregulated (38% higher than at device implantation), and Ca2+ in sarcoplasmatic reticulum was increased. Terracciano et al. have shown that mechanical unloading is associated with modifications in excitation-contraction coupling, and sarcoplasmatic Ca2+ homeostasis (8).
In a rat model, protracted ventricular unloading produces depressed myocardial contractility. The mechanisms of cardiomyocyte contractile and relaxation impairment, in this case, are due to impaired myofilament sensitivity to Ca2+ and reduced SERCA2a uptake, respectively (9).
The advantages of unloading on calcium handling and contractility could be time-dependent. Patients with a shorter duration of mechanical support (10). The phenomenon of calcium handling and contractility is not constant throughout the duration of unloading. This may suggest it is possible to predict a time-dependent recovery in early phases of ventricular unloading or it could be an indication of adverse remodelling occurring in later stages of unloading.
Apoptosis
LVAD mechanical support is associated with a decrease in apoptosis, which is an important contributor to HF.
NF-κB is a transcription factor implicated in immune and inflammatory responses, developmental processes, cellular growth, and apoptosis. In particular NF-κB regulates the expression of genes encoding for tumor necrosis factor (TNF) α, interleukin (IL)-6 and hemeoxygenase (11). TNFα leads to the recruitment of Caspase death proteases, and a significant decrease in TNFα levels in the myocardium of patients supported by LVAD has been demonstrated (12).
Hemeoxygenase-1 (HO-1, HSP 32) is an inducible enzyme isoform in response to stress and also possesses an anti-apoptotic function. In HF, HO-1 is significantly increased, but after unloading, the HO-1 expression normalizes (13-15).
Increasing stem cell recruitment and proliferation
After unloading, a significant decrease in mean cardiomyocyte DNA content and an increase in numbers of binucleated cardiomyocytes have been demonstrated, suggesting a numerical increase of cardiomyocytes. Wohlschlaeger et al. propose that the vast polyploidy of cardiomyocytes in HF results from hypertrophic growth associated with repeated rounds of DNA synthesis with an incomplete attempt to enter mitosis. After LVAD unloading, the hypertrophic stimulation is decreased, so the brake inhibiting entry into mitosis is released, resulting in cell duplication with formation of binucleated cardiomyocytes. The decrease in mean DNA content in cardiomyocytes suggests that stem and progenitor cell proliferation occurs after unloading (16). The heart contains a reservoir of stem and progenitor cells, including c-kit (CD117) stem cells and side population cells (SPC). After LVAD unloading there is a significant increase of c-kit+/MEF-2+ cells, suggestive of migration and/or proliferation of SPCs of unknown origin (17). A pool of stem cells is released from the bone marrow. Manginas et al., demonstrate that a transient increase occurs in the number of circulating CD34+ cells after LVAD implantation (18). Animal models (heterotopic heart unloaded in rats) are consistent with these findings and provide validation that hemodynamic unloading improves the regeneration of myocytes in the failing heart by increasing cell proliferation, inhibiting cell apoptosis, and improving stem-cell recruitment (19).
Remodelling of cardiac mitochondria
Functional and structural derangement of myocardial mitochondria may contribute to HF (20). Mitochondrial respiratory function was evaluated with polarographic techniques both in HF due to ICD and DCD and, following mechanical unloading, mitochondrial function of cardiomyocytes was improved. One possible mechanism for improvement in the respiratory function is an alteration in mitochondrial calcium levels, which could be linked with calcium handling. Thus respiratory control diminishes as cytosolic calcium overload occurs (20). Furthermore, mechanical LVAD support increases endogenous NO-mediated regulation of mitochondrial respiration (21).
HF produces significant structural variations in myocardial mitochondria cardiolipin content both in ICD and DCD, but alters cardiolipin composition only in ICD. Efficiency of mitochondrial coupling is largely determined by the functional status of the inner membranes, of which cardiolipin is the characteristic phospholipid. LVAD support does not restore cardiolipin content of failing hearts, but does normalize cardiolipin composition in those with ICD (22).
The sympathetic nervous system
β-adrenergic receptor (β-AR) signaling can be altered by: (I) receptor downregulation; (II) receptor desensitization. β-AR in the heart are functionally coupled with G protein-adenylylcyclase signal transduction cascade and serve as targets of β-ARK and protein kinase A (PKA). β-ARK leads to desensitization of the receptor by uncoupling the receptors from the stimulatory G protein Gs. The cAMP-PKA phosphorylates several proteins related to excitation-contraction coupling such as activation of L-Type Calcium Channels (CaCn), of SERCA2, increasing reuptake of Ca2+. There is a link between sympathetic nervous system and calcium handling.
β-AR have demonstrated desensitization in patients with chronic HF, mediated by β-ARK from epinephrine overstimulation. Thus β-AR are also downregulated by decreased synthesis or by phosphoinositide 3-kinase (PI3K)-gamma with redistribution of β-receptors into endosomal compartments (23). Mechanical unloading with LVAD support is associated with reversal of this β-receptor desensitization and downregulation and it is accompanied by increased beta adrenergic receptor density (24).
Extracellular matrix (ECM)
The ECM provides the scaffold necessary for the alignment of myocardial cells within the myocardium. ECM turnover, with an increase in fibrosis, is a hallmark feature of myocardial remodelling in chronic HF and results from an imbalance of matrix metalloproteinases (MMPs) and their inhibitors, the tissue inhibitors of metalloproteinases (TIMPs).
The most abundant proteins are collagens types I and III and the role of changing metabolic responses of collagen concentration and crosslinking, after LVAD support, remains controversial. During the matrix remodelling process, type I and III procollagen is cleaved to form type I and III collagen and their amino terminal propeptides of type I and III procollagen (PINP and PIIINP). After LVAD support, Klotz et al. demonstrated an increase in collagen cross-linking and the ratio of collagen type I to III and therefore an increase in myocardial stiffness (25). Xydas et al. on the other hand showed a decrease in collagen deposition from time of LVAD implantation to time of transplantation in a group of patients who were bridged to transplantation (26). Similarly, Bruckner et al. showed that long-term mechanical circulatory support significantly reduced collagen content and fibrosis (27). Bruggink et al. suggested a biphasic pattern for the collagen turnover and the volume of ECM with an initial increase in fibrosis, followed by a subsequent regression after 200 days of LVAD support (28).
A histological study from autopsy specimens of ventricular apical core biopsies and explanted hearts, demonstrated that LVAD led to significant improvement in several forms of myocyte damage (coagulative necrosis, myocytolysis and myocyte waviness) but not to significant reduction in scores for fibrosis, increased eosinophilia or contraction band necrosis (29).
Matrix metalloproteinases (MMPs)
MMPs constitute a group of zinc- and calcium-dependent endopeptidases, which are able to degrade ECM components and modulate the function of a variety of endogenous proinflammatory and vasoactive molecules. More than 20 MMPs have been described. The failing heart displays an increased expression of MMP-2 on mRNA and protein levels. The prognosis of HF patients seems to correlate with the severity of NYHA class, and plasma levels of MMP-2 (30). However, the implantations of LVADs or total artificial heart (TAH) were not shown to influence MMP-2 plasma concentration (31). Nevertheless, Li et al. (32) studied MMP-9 activity in a group of patients suffering from DCM or ICM at the time of LVAD implantation and again at explantation of the device. The MMP-9 activity was decreased in patients post-LVAD compared with baseline activity. In addition, the pro-MMP-9 tissue content was significantly decreased by LVAD. This could suggest that cardiac unloading by LVAD leads to reduced ECM degradation in the myocardium.
Tissue inhibitors of metalloproteinases (TIMPs)
The activity of MMPs is controlled by expression and secretion of the TIMPs. A trial has shown that the plasma level of TIMP-1 was higher in LVAD patients at device implantation compared with healthy controls, with no changes after unloading of the heart by the device. Interestingly, in TAH patients, TIMP-1 plasma levels were significantly increased at implantation and remain elevated after implantation of the TAH. This trial demonstrated that plasma concentrations of TIMP-1 were not regulated during mechanical unloading, and that the myocardium is not the only source of circulating TIMP-1, reflecting a systemic remodelling (31).
Two studies by Felkin et al. investigated the expression of TIMP-4 mRNA in the myocardium of DCM patients. Symptomatic DCM patients who required LVAD unloading displayed higher TIMP-4 mRNA expression than individuals with stable DCM undergoing HTx without prior VAD (33). In a second trial, TIMP-4 expression was assessed at LVAD implantation in advanced DCM patients and again at explantation. TIMP-4 was expressed at significantly lower levels at explantation of the device (34). However, by contrast, Li et al. (32) found TIMP-4 protein was unchanged during VAD support. Thus there is a discordance in mRNA and protein expression of TIMP-4 during mechanical unloading.
Osteopontin (OPN)
OPN is a multifunctional protein, involved in diverse biological processes such as a protein anchoring, biomineralization, immunoregulation, leukocyte recruitment, and regulation of cell adhesion and cell survival. Levels of OPN mRNA in the myocardium of HF patients showed a significant decrease after LVAD support, but OPN protein expression was not decreased (35). Significant elevation of OPN levels before LVAD placement is associated with right ventricular failure (RVF) development. Persistent elevation of circulating ECM markers after LVAD implantation may be characteristic of patients who develop RVF. OPN levels >260 ng/mL discriminate patients who develop RVF after LVAD implantation (36).
The neurohormonal and cytokine network
HF is characterized by neurohormonal and inflammatory cytokine activation, influencing heart contractility and filling, promoting apoptosis, and contributing to the process of myocardial remodelling. Several studies have shown elevated levels of inflammatory cytokines such as TNFα, IL-1β and IL-6 in HF patients in plasma and circulating leukocytes, as well as in the failing myocardium itself. Neurohormonal systems (catecholamines, natriuretic peptides and components of the renin-angiotensin axis) are increased in HF and have been found to have pathophysiological and prognostic implications, because some of these molecules exert local paracrine activation (1).
Brain natriuretic peptide (BNP) and NT-proBNP
BNP and its amino terminal cleavage fragment (NT-proBNP) are peptide hormones released from cardiomyocytes under mechanical stress. Postoperative BNP levels have been shown to decrease dramatically with the initiation of LVAD support. These levels correlated inversely with the degree of hemodynamic support rendered at various settings of the number of revolutions per minute (RPM). Overall, BNP levels decreased significantly two days after RPM increase. There was a significant inverse association between the postoperative BNP levels and the degree of LVAD support (37).
The important reduction in serum BNP concentration after mechanical support coincides with a decrease in BNP mRNA and protein expression in the heart (38). Mechanical unloading of the heart or removal of the failing ventricles reduced BNP plasma concentrations both in LVAD and in TAH patients (31).
Galectin-3 (Gal-3)
Gal-3 is a profibrotic protein, beta-galactosidase binding lectin, secreted by activated macrophages, and has a role in development of fibrosis in injured myocardial tissue. Gal-3 displays several autocrine and paracrine effects and could improve pro-inflammatory networks. In patients with HF, a single level of Gal-3 predicts mortality at four years, independently of echocardiographic signs of disease severity (39). Patients not surviving with mechanical support presented significantly high plasma concentrations of Gal-3 at the time of LVAD implantation compared with those patients who were transplanted (31).
Erkilet et al. analyzed a cohort of 151 patients and demonstrated that a higher Gal-3 level pre-LVAD implantation, was associated with increased mortality risk during mechanical support. These data deserve further investigation, since Gal-3 might be a useful biomarker for prediction of mortality in severe HF patients, planned for LVAD support (40).
ST2
ST2 is a member of the interleukin-1 receptor family, and is secreted by both fibroblasts and cardiomyocytes in response to strain. ST2 exists in a membrane-bound receptor and in a truncated soluble form (sST2), measurable in the blood of patients after myocardial infarction and in those with HF. ST2 belongs to a cardioprotective signaling system consisting of paracrine interactions between IL-33, produced by cardiac fibroblasts and transmembrane ST2 receptors on cardiac myocytes. IL-33 neutralizes circulating sST2 and has been associated with a decrease in myocardial strain.
Ky et al. (41), reported that ST2 level is strongly correlated with worse HF, and patients with high levels of circulating sST2 had an elevated increased risk of death or heart transplantation. Assessment of ST2 may make a customized risk stratification in patients with HF possible, allowing identification of those who need more aggressive treatment strategies.
Values of circulating sST2 in patients at LVAD explantation were lower compared to the time of mechanical support implantation; conversely, there was no significant change in IL-33 level. The IL-33/ST2 ratio rises after LVAD support. The increase in IL-33 relative to sST2 after LVAD unloading may reflect normalization of cardioprotective signaling (sST2/IL33) with normalization of cardiac filling and ventricular contraction (42).
Growth differentiation factor-15 (GDF-15)
GDF-15 is a cytokine secreted in stress-response situations such as oxidative stress, inflammation or tissue injury. Increased circulating levels of GDF-15 have been linked to the incidence and prognosis of HF. Elevated circulating levels of GDF-15 in patients with end-stage DCM correlate with myocardial fibrosis and kidney function and is significantly reduced after one month of LVAD unloading, remaining constant thereafter. The heart is not an important source of GDF-15 production in these patients, because there is very little protein expression and mRNA of GDF-15 in the myocardium (43).
MicroRNA (miRNA)
miRNAs comprise short, non-coding RNAs, which act as intracellular endogenous RNAs to control gene expression on a post-translational level. miRNAs can be detected in myocardial samples or in a circulating form. In a recent review, seven circulating miRNAs were validated in the plasma of patients with HF (miR-423-5p, miR-18b*, miR-129-5p, miR-1254, miR-675, HS_202.1, and miR-622).
In this group of miRNAs, the most strongly related to the clinical diagnosis of HF was miR-423-5p (44). Functional myocardial response to LVAD unloading (EF >50% and a relative decrease in LV end-systolic volumes >25%) was associated with lower circulating levels of miRs-15b and -133b. The expression of miRs-208; -499; -205; -133a; -145; -21 and -29a were not significantly changed during LVAD with or without functional recovery (45). Therefore, it remains to be determined whether circulating miRNA has a biological function and modulates pattern of gene expression.
Other studies evaluated miRNA from myocardial samples. Matkovich et al., demonstrated miRNAs associated with HF (miR-24, miR-125b and miR-19) and their reversibility after LVAD support (46). Low level of miRs-23a and -195 in cardiac expressions and smaller cardiomyocyte size at the time of LVAD implantation were associated with LV functional recovery (47).
In the end, the spectrum of miRNA is wide and includes both circulating miRNA, and miRNA expressed in the myocardium. These preliminary studies may demonstrate differential expression of miRNA during LVAD unloading, which could be used as markers to assess for possible recovery and investigate the functional genetic pathway that correlates with clinical recovery.
Heart valve
Increasing assist device flows in low cardiac function resulted in transvalvular high pressures above the aortic leaflets. This condition maintains the aortic valve closed throughout cardiac cycle and has been associated with impaired leaflet function, aortic root dilatation and aortic valve fusion and/or incompetence. The average leaflet strain is increased during LVAD support, due to the physiologic strain during ventricular systole and a continuous flow strain during diastole by mechanical support. This mechanism can explain the structural changes of the aortic valve during LVAD unloading (48,49). Acquired aortic stenosis of the native aortic valve and thrombotic occlusion of a prosthetic aortic valve in the native heart have both been demonstrated after autopsy of patients with VAD (29). Furthermore, secondary severe aortic insufficiency can become a significant complication. Recently, percutaneous transcatheter closure with the Amplatzer cribriform device (AGA Medical, Plymouth, Minnesota, USA) of the aortic valve was shown to effectively treat LVAD-associated aortic insufficiency and reduce pulmonary capillary wedge pressure (50).
Future perspectives
The cardiac remodelling mediated by LVAD must be contextualized within the etiology of cardiomyopathy, the duration of the unloading and right HF. It is important to differentiate between ICD and DCD with genetic mutations. DCD with abnormal myocardial proteins cannot undergo recovery with LVAD support, because there is an intrinsic abnormality of the genome. Stem cells of patients with genetic DCD themselves possess the defect. ICD hearts could achieve a greater degree of myocardial recovery because their cardiac stem cells have no intrinsic mutation. For this reason, after unloading it is plausible that the cardiomyocytes in ICD recover more than the DCD.
It is also difficult to compare cardiac fibrosis between ICD and DCD, as the first is mediated by a process of ischemia-necrosis-fibrosis, and geographically distributes downstream of the coronary stenosis. Fibrosis in DCD is mediated by a process of myocyte failing-apoptosis-fibrosis and is distributed ubiquitously in the myocardium. It is therefore important to differentiate the etiology of cardiomyopathy to assess the changes after LVAD unloading and further studies will therefore be required. This view is consistent with disease-specific reverse remodelling of heart after LVAD support in ICD and DCD.
The time of unloading is important because there are time-related cardiac changes. In the first phase there is an improvement and normalization of the systems previously discussed. Subsequently, there has been shown to be a decrease in the concentration of sarcoplasmic calcium, reducing the capacity for excitation-contraction coupling of myocardial cells. Hence, it seems there is a window period (about 30 days) in which the myocardium can undergo recovery.
The duration of unloading could trigger differing activation of the neurohormonal and cytokine network, mediated mainly by the transition from pulsatile flow to continue. Others studies will be required to determine the mechanisms and pathways involved. Additionally, after a long period of unloading of the left ventricle, a frequent complication is RVF. In this case, all the aforementioned mechanisms of HF could recommence, and for this reason, some biochemical markers may rise.
Translational research in LVAD unloading is an emerging area that has provided new information on signaling pathways of reverse remodelling after HF and helped to develop new therapeutic targets and strategies to induce further significant reverse remodelling and durable myocardial recovery.
Acknowledgements
Disclosure: The authors declare no conflict of interest.
References
- Yancy CW, Jessup M, Bozkurt B, et al. 2013 ACCF/AHA guideline for the management of heart failure: a report of the American College of Cardiology Foundation/American Heart Association Task Force on Practice Guidelines. Circulation 2013;128:e240-327. [PubMed]
- Barbone A, Holmes JW, Heerdt PM, et al. Comparison of right and left ventricular responses to left ventricular assist device support in patients with severe heart failure: a primary role of mechanical unloading underlying reverse remodeling. Circulation 2001;104:670-5. [PubMed]
- Maybaum S, Mancini D, Xydas S, et al. Cardiac improvement during mechanical circulatory support: a prospective multicenter study of the LVAD Working Group. Circulation 2007;115:2497-505. [PubMed]
- Dipla K, Mattiello JA, Jeevanandam V, et al. Myocyte recovery after mechanical circulatory support in humans with end-stage heart failure. Circulation 1998;97:2316-22. [PubMed]
- Ambardekar AV, Walker JS, Walker LA, et al. Incomplete recovery of myocyte contractile function despite improvement of myocardial architecture with left ventricular assist device support. Circ Heart Fail 2011;4:425-32. [PubMed]
- Birks EJ, Hall JL, Barton PJ, et al. Gene profiling changes in cytoskeletal proteins during clinical recovery after left ventricular-assist device support. Circulation 2005;112:I57-64. [PubMed]
- Arai M, Alpert NR, MacLennan DH, et al. Alterations in sarcoplasmic reticulum gene expression in human heart failure. A possible mechanism for alterations in systolic and diastolic properties of the failing myocardium. Circ Res 1993;72:463-9. [PubMed]
- Terracciano CM, Koban MU, Soppa GK, et al. The role of the cardiac Na+/Ca2+ exchanger in reverse remodeling: relevance for LVAD-recovery. Ann N Y Acad Sci 2007;1099:349-60. [PubMed]
- Soppa GK, Lee J, Stagg MA, et al. Prolonged mechanical unloading reduces myofilament sensitivity to calcium and sarcoplasmic reticulum calcium uptake leading to contractile dysfunction. J Heart Lung Transplant 2008;27:882-9. [PubMed]
- Ogletree ML, Sweet WE, Talerico C, et al. Duration of left ventricular assist device support: Effects on abnormal calcium cycling and functional recovery in the failing human heart. J Heart Lung Transplant 2010;29:554-61. [PubMed]
- Bartling B, Milting H, Schumann H, et al. Myocardial gene expression of regulators of myocyte apoptosis and myocyte calcium homeostasis during hemodynamic unloading by ventricular assist devices in patients with end-stage heart failure. Circulation 1999;100:II216-23. [PubMed]
- Schumann H, Morawietz H, Hakim K, et al. Alternative splicing of the primary Fas transcript generating soluble Fas antagonists is suppressed in the failing human ventricular myocardium. Biochem Biophys Res Commun 1997;239:794-8. [PubMed]
- Grabellus F, Levkau B, Sokoll A, et al. Reversible activation of nuclear factor-kappaB in human end-stage heart failure after left ventricular mechanical support. Cardiovasc Res 2002;53:124-30. [PubMed]
- Torre-Amione G, Stetson SJ, Youker KA, et al. Decreased expression of tumor necrosis factor-alpha in failing human myocardium after mechanical circulatory support: A potential mechanism for cardiac recovery. Circulation 1999;100:1189-93. [PubMed]
- Grabellus F, Schmid C, Levkau B, et al. Reduction of hypoxia-inducible heme oxygenase-1 in the myocardium after left ventricular mechanical support. J Pathol 2002;197:230-7. [PubMed]
- Wohlschlaeger J, Levkau B, Brockhoff G, et al. Hemodynamic support by left ventricular assist devices reduces cardiomyocyte DNA content in the failing human heart. Circulation 2010;121:989-96. [PubMed]
- Wohlschlaeger J, Levkau B, Takeda A, et al. Increase of ABCG2/BCRP+ side population stem cells in myocardium after ventricular unloading. J Heart Lung Transplant 2012;31:318-24. [PubMed]
- Manginas A, Tsiavou A, Sfyrakis P, et al. Increased number of circulating progenitor cells after implantation of ventricular assist devices. J Heart Lung Transplant 2009;28:710-7. [PubMed]
- Suzuki R, Li TS, Mikamo A, et al. The reduction of hemodynamic loading assists self-regeneration of the injured heart by increasing cell proliferation, inhibiting cell apoptosis, and inducing stem-cell recruitment. J Thorac Cardiovasc Surg 2007;133:1051-8. [PubMed]
- Lee SH, Doliba N, Osbakken M, et al. Improvement of myocardial mitochondrial function after hemodynamic support with left ventricular assist devices in patients with heart failure. J Thorac Cardiovasc Surg 1998;116:344-9. [PubMed]
- Mital S, Loke KE, Addonizio LJ, et al. Left ventricular assist device implantation augments nitric oxide dependent control of mitochondrial respiration in failing human hearts. J Am Coll Cardiol 2000;36:1897-902. [PubMed]
- Heerdt PM, Schlame M, Jehle R, et al. Disease-specific remodeling of cardiac mitochondria after a left ventricular assist device. Ann Thorac Surg 2002;73:1216-21. [PubMed]
- Perrino C, Schroder JN, Lima B, et al. Dynamic regulation of phosphoinositide 3-kinase-gamma activity and beta-adrenergic receptor trafficking in end-stage human heart failure. Circulation 2007;116:2571-9. [PubMed]
- Pandalai PK, Bulcao CF, Merrill WH, et al. Restoration of myocardial beta-adrenergic receptor signaling after left ventricular assist device support. J Thorac Cardiovasc Surg 2006;131:975-80. [PubMed]
- Klotz S, Foronjy RF, Dickstein ML, et al. Mechanical unloading during left ventricular assist device support increases left ventricular collagen cross-linking and myocardial stiffness. Circulation 2005;112:364-74. [PubMed]
- Xydas S, Rosen RS, Ng C, et al. Mechanical unloading leads to echocardiographic, electrocardiographic, neurohormonal, and histologic recovery. J Heart Lung Transplant 2006;25:7-15. [PubMed]
- Bruckner BA, Stetson SJ, Perez-Verdia A, et al. Regression of fibrosis and hypertrophy in failing myocardium following mechanical circulatory support. J Heart Lung Transplant 2001;20:457-64. [PubMed]
- Bruggink AH, van Oosterhout MF, de Jonge N, et al. Reverse remodeling of the myocardial extracellular matrix after prolonged left ventricular assist device support follows a biphasic pattern. J Heart Lung Transplant 2006;25:1091-8. [PubMed]
- Rose AG, Park SJ. Pathology in patients with ventricular assist devices: a study of 21 autopsies, 24 ventricular apical core biopsies and 24 explanted hearts. Cardiovasc Pathol 2005;14:19-23. [PubMed]
- Ahmed SH, Clark LL, Pennington WR, et al. Matrix metalloproteinases/tissue inhibitors of metalloproteinases: relationship between changes in proteolytic determinants of matrix composition and structural, functional, and clinical manifestations of hypertensive heart disease. Circulation 2006;113:2089-96. [PubMed]
- Milting H, Ellinghaus P, Seewald M, et al. Plasma biomarkers of myocardial fibrosis and remodeling in terminal heart failure patients supported by mechanical circulatory support devices. J Heart Lung Transplant 2008;27:589-96. [PubMed]
- Li YY, Feng Y, McTiernan CF, et al. Downregulation of matrix metalloproteinases and reduction in collagen damage in the failing human heart after support with left ventricular assist devices. Circulation 2001;104:1147-52. [PubMed]
- Felkin LE, Birks EJ, George R, et al. A quantitative gene expression profile of matrix metalloproteinases (MMPS) and their inhibitors (TIMPS) in the myocardium of patients with deteriorating heart failure requiring left ventricular assist device support. J Heart Lung Transplant 2006;25:1413-9. [PubMed]
- Felkin LE, Lara-Pezzi E, George R, et al. Expression of extracellular matrix genes during myocardial recovery from heart failure after left ventricular assist device support. J Heart Lung Transplant 2009;28:117-22. [PubMed]
- Schipper ME, Scheenstra MR, van Kuik J, et al. Osteopontin: a potential biomarker for heart failure and reverse remodeling after left ventricular assist device support. J Heart Lung Transplant 2011;30:805-10. [PubMed]
- Kato TS, Chokshi A, Singh P, et al. Markers of extracellular matrix turnover and the development of right ventricular failure after ventricular assist device implantation in patients with advanced heart failure. J Heart Lung Transplant 2012;31:37-45. [PubMed]
- Sareyyupoglu B, Boilson BA, Durham LA, et al. B-type natriuretic peptide levels and continuous-flow left ventricular assist devices. ASAIO J 2010;56:527-31. [PubMed]
- Bruggink AH, de Jonge N, van Oosterhout MF, et al. Brain natriuretic peptide is produced both by cardiomyocytes and cells infiltrating the heart in patients with severe heart failure supported by a left ventricular assist device. J Heart Lung Transplant 2006;25:174-80. [PubMed]
- Shah RV, Chen-Tournoux AA, Picard MH, et al. Galectin-3, cardiac structure and function, and long-term mortality in patients with acutely decompensated heart failure. Eur J Heart Fail 2010;12:826-32. [PubMed]
- Erkilet G, Schulte-Eistrup S, Morshuis M, et al. Plasma galectin 3 is increased in terminal heart failure patients and is elevated in patients not surviving mechanical circulatory support. J Heart Lung Transplant 2010;29:S65.
- Ky B, French B, McCloskey K, et al. High-sensitivity ST2 for prediction of adverse outcomes in chronic heart failure. Circ Heart Fail 2011;4:180-7. [PubMed]
- Qiu S, Pronschinske K, Wu C, et al. The Impact of Mechanical Unloading Through Ventricular Assist Device Placement on Cardiac ST2/IL-33 Signaling in Patients with Advanced Heart Failure. Circulation 2011;124:A13781.
- Lok SI, Winkens B, Goldschmeding R, et al. Circulating growth differentiation factor-15 correlates with myocardial fibrosis in patients with non-ischaemic dilated cardiomyopathy and decreases rapidly after left ventricular assist device support. Eur J Heart Fail 2012;14:1249-56. [PubMed]
- Creemers EE, Tijsen AJ, Pinto YM. Circulating microRNAs: novel biomarkers and extracellular communicators in cardiovascular disease? Circ Res 2012;110:483-95. [PubMed]
- Galenko O, Kfoury AG, Selzman CH, et al. LVAD-Induced Improvement in Myocardial Function Is Associated with a Unique Pattern of Circulating microRNAs. J Heart Lung Transplant 2013;32:S148.
- Matkovich SJ, Van Booven DJ, Youker KA, et al. Reciprocal regulation of myocardial microRNAs and messenger RNA in human cardiomyopathy and reversal of the microRNA signature by biomechanical support. Circulation 2009;119:1263-71. [PubMed]
- Ramani R, Vela D, Segura A, et al. A micro-ribonucleic acid signature associated with recovery from assist device support in 2 groups of patients with severe heart failure. J Am Coll Cardiol 2011;58:2270-8. [PubMed]
- May-Newman K, Enriquez-Almaguer L, Posuwattanakul P, et al. Biomechanics of the aortic valve in the continuous flow VAD-assisted heart. ASAIO J 2010;56:301-8. [PubMed]
- Tuzun E, Pennings K, van Tuijl S, et al. Assessment of aortic valve pressure overload and leaflet functions in an ex vivo beating heart loaded with a continuous flow cardiac assist device. Eur J Cardiothorac Surg 2014;45:377-83. [PubMed]
- Parikh KS, Mehrotra AK, Russo MJ, et al. Percutaneous transcatheter aortic valve closure successfully treats left ventricular assist device-associated aortic insufficiency and improves cardiac hemodynamics. JACC Cardiovasc Interv 2013;6:84-9. [PubMed]